The St. Johns River Water Management District maintains data on springs and spring flows for water resource protection and long-term water supply planning. Water level, quality and discharge data obtained from monitoring networks increase scientists’ understanding of the hydrogeologic, climatic and human factors that affect water resources.
How often monitoring is conducted is unique to each spring and is contingent on many factors. In some cases, larger springs on public property may be sampled every few months, while smaller springs on public or private property are not regularly monitored. Historical spring flow data has been collected by the U.S. Geological Survey since 1929 and by the water management district since 1984.
The monitoring data presented on these web pages is intended to provide general information about springs. The data reflects information compiled in 2010. While the district collects data on a continual basis, these pages are updated every five years.
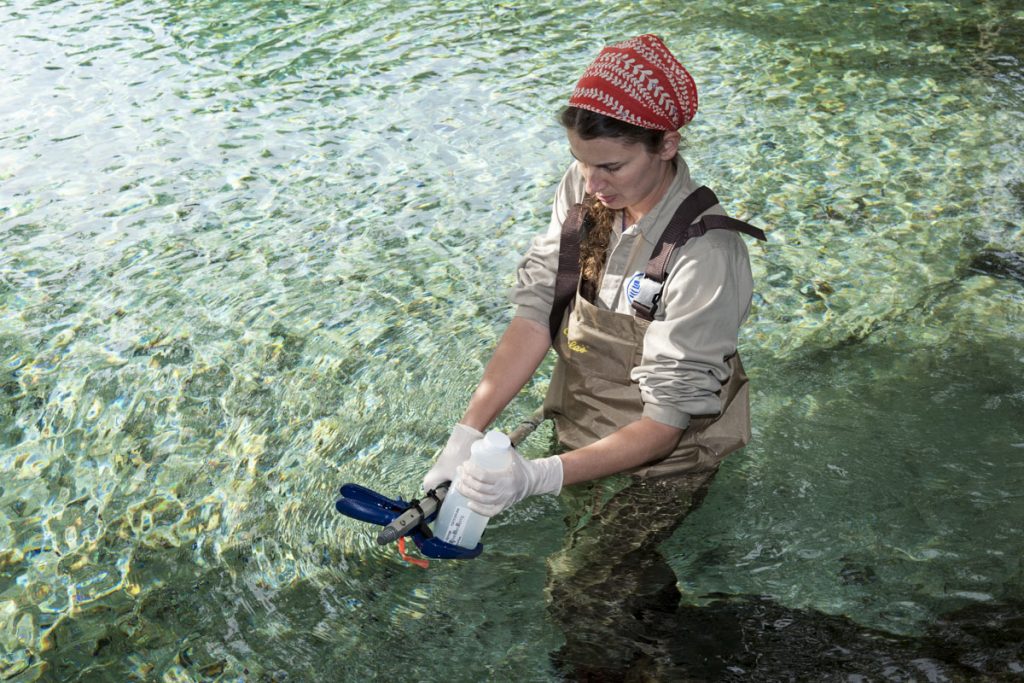
Frequently Asked Questions
Field staff frequently are asked questions when doing their work in the field. Following are some commonly asked questions they receive while doing their work at a spring.
What are you doing?
Staff are measuring the flow of water (discharge) exiting the spring.
To measure flow, staff use an Acoustic Doppler Current Profiler, or ADCP. It consists of sonar sensors that measure the water’s velocity, direction and water depth at multiple locations across the steam and at multiple depths. The District then combines all the measurements to estimate the stream’s entire discharge.
How do you use the ADCP to measure discharge?
The ADCP is mounted on a small watercraft and pulled from one side of the spring run to the other side along a line, or cross-section. As the ADCP travels the cross-section, the sensors continuously measure the water’s velocity, direction and depth, so that a “profile” of the flow is constructed. Due to turbulence in the flow, sometimes it takes several measurements before an acceptable set of measurements are obtained. A typical ADCP measurement is illustrated below. This is time consuming, technical and complex field work.
Back in the office, the Data Collection Specialists process the data using special software to calculate the total spring run’s discharge (flow rate in cubic feet per second). They carefully review the results, applying their extensive experience to ensure the data are accurate.
Can you take the measurement anywhere on the spring run?
Because there is turbulence in the flow, the best measurement is done where the flow is concentrated, and where the spring run banks and bottom are smoother, with the least amount of irregularity, blockages and vegetation. Staff also like to find cross-sections that are more stable over time.
Are you allowed to be there?
Yes, the St. Johns River Water Management District has the mission to monitor natural systems, such as springs, rivers and lakes. We have obtained permission from the property owner to take discharge measurements.
Do the springs fluctuate much?
Yes, the elevation of spring runs and their discharge rate fluctuates depending on rainfall, tailwater conditions and groundwater withdrawal within the springshed.
How does the District use the data that is collected?
The data are collected for water supply planning, regulatory determination, groundwater and surface water modeling, flood protection and aquatic restoration projects.
Can you drink the water that comes out of the springs?
Water must be treated to meet potable (drinking) water quality standards before it is suitable for human consumption.
How frequently do you measure the flow?
Bi-monthly. Staff visit routinely so that we can continuously estimate discharge by continuously measuring the water’s elevation using a permanent gauge station which collects and stores data when we are not here.
How much flow is there and where can I find the data you collect?
The raw data collected in the field are processed in the office. Then the data are separately quality assured, stored in a District database, and published on the District’s website at www.sjrwmd.com/data/hydrologic and http://webapub.sjrwmd.com/agws10/hdsnew/map.html for near real-time data.
The amount of water that flows from a spring depends on several factors, including rainfall, size of the caverns within the rocks, the water pressure in the aquifer, and the size of spring recharge basin. Human activities can also influence the volume of water that discharges from a spring; for example, increased groundwater withdrawals can reduce the hydraulic pressure in an aquifer, causing water levels to decline and spring flows to decrease.
Historical spring flow data has been collected by the U.S. Geological Survey since 1929 and by the St. Johns River Water Management District since 1984. The amount of discharge data collected at each spring varies from only one measurement in several of the smaller springs to several hundred measurements taken at the first magnitude springs.
Find discharge information for specific springs in the biannual spring discharge report
Spring water quality
Accurate and reliable water quality data are required to characterize groundwater chemistry for use in resource management. Major ion chemistry is used as a general indicator of groundwater quality and provides a means to identify regional differences and changes in water quality with time. A spring’s water quality is determined by several factors. These include the chemical composition of the water entering the aquifer, the composition and solubility of the rocks with which the water comes into contact along flow paths, the length of time the water is in contact with the rocks as it moves from recharge to discharge areas, and the mixing of fresh groundwater with residual formation water or seawater.
Land use activities in a spring’s recharge basin and the upconing of poorer quality water from deeper zones due to groundwater withdrawals may also impact water quality. The most pristine springs in the state occur in the Ocala National Forest, with spring recharge basins that encompass limited land uses. Other springs have recharge basins that encompass urban land uses and well withdrawals that have resulted in spring flow reductions and declining water quality.
The chemical composition and physical properties of a spring sample represent the net effect of all the previous chemical processes that have dissolved, altered, or precipitated the chemical constituents. The major constituents considered are those commonly present in concentrations exceeding 1.0 milligram per liter and that constitute a major part of the total dissolved solids content. The major cations include calcium, magnesium, sodium, and potassium. The major anions include chloride, and sulfate, carbonate alkalinity. Fluoride, nitrate + nitrite, and orthophosphate are important minor anions. Chemically related parameters include total dissolved solids and total organic carbon. Field measurement data include temperature, specific conductance, pH, and spring discharge.
The water quality status and trends report provides the current statistical analysis.
Definitions of water quality variables
Alkalinity
Alkalinity is an indicator of the capacity of solutes in water to react with and neutralize acid. Alkalinity is produced by the reaction of carbon dioxide and water in the atmosphere or the soil zone, the dissolution of calcite and dolomite, and the oxidation of organic materials by microorganisms. Bicarbonate is the dominant anion in natural waters and is the dominant constituent of alkalinity. As reported for the spring data, alkalinity (as CaCO3) includes bicarbonate (HCO3–), carbonate (CO32–), and other anions that can be neutralized by a strong acid.
Calcium
The calcium ion (Ca2+) is dissolved from most soils and rocks, and especially from limestone, dolomite, and gypsum. Calcium is a dominant cation resulting from the chemical weathering of the mineral calcite (CaCO3) in limestone and the mineral dolomite (CaMg(CO3)) in dolostone. Calcium is also present in large concentrations in some brines, seawater, and connate water. The concentration of dissolved calcium is a result of the water’s contact with aquifer materials, residence time, and flow path, with highest concentration in alkaline waters that are in equilibrium with aquifer materials. Calcium causes water to be hard and contributes to the scale-forming properties of water.
Chloride
The sources of chloride (Cl–) in Florida’s aquifer systems are the lateral intrusion of recent seawater, residual seawater in an aquifer, and marine aerosols. Chloride is a relatively inert element that does not readily enter into mineral reactions, so the occurrence of chloride is useful for identifying laterally intruded seawater and mixing of fresh groundwater with residual seawater. Concentrations above 300 mg/L in combination with sodium give a salty taste to water. The drinking water standard for chloride is 250 mg/L.
Fluoride
Fluoride (F–) in Florida’s aquifer systems is derived mostly from the weathering of carbonate-fluorapatite in the Hawthorn Group, indicating that the water has come into contact with the Hawthorn Group at some time in the past, or from the mixing of seawater with groundwater. The drinking water standard for fluoride, which addresses toxicity, is 2.0 mg/L. In small amounts of less than 1.0 mg/L, fluoride is considered beneficial for preventing cavities.
Magnesium
Magnesium (Mg2+) is dissolved from practically all soils and rocks, especially magnesium-rich clays in the Hawthorn Group and dolomite in the Floridan aquifer. Magnesium is present in large concentrations in some brines, seawater, and connate water. It causes water to be hard and contributes to the scale-forming properties of water.
Nitrate + Nitrite
Nitrogen compounds occur in groundwater as a result of specific land uses, the leaching of organic soils, and from precipitation. Sources of nitrogen from man’s activities include agricultural fertilizers, animal wastes, and human wastes. Nitrogen is transformed between organic nitrogen (TKN), ammonium (NH4+), nitrite (NO2–), nitrate (NO3–) and other nitrogen compounds depending on oxidation/reduction conditions, microbial activity, and plant utilization. Nitrate is the stable form of nitrogen in oxidizing environments. Nitrite is unstable in the presence of oxygen and is present in small concentrations in most waters. Once nitrate enters the aquifer and is isolated from environments where denitrification and plant fixation occur, nitrate behaves more-or-less conservatively and can move long distances in aquifers. The drinking water standard for nitrate is 10 mg/L as nitrogen, which is intended to protect human health and is not based on the protection of ecological systems. Elevated nutrient concentrations may lead to increases in algae growth, which decreases water clarity and changes the aesthetic qualities and ecology of springs.
Phosphorus / Orthophosphate
Sources of phosphate (orthophosphate, PO43–) in groundwater include the dissolution of phosphate-rich sediments, leaching from organic sediments and plant materials, agricultural fertilizers, human waste effluent (such as from septic tank systems), industrial effluent, and other waste disposal practices. The orthophosphate ion is soluble in acidic waters, such as occur in siliciclastic horizons of the surficial aquifer system, but insoluble in alkaline aquifers, such as occur in the Floridan aquifer. In carbonate-rich aquifers, orthophosphate is removed by precipitation of carbonate-hydroxylapatite and alkaline waters seldom have detectable phosphate as a result.
pH
The variable pH reflects the potential for acid-base reactions in water. The pH of aquifer water is a result of past chemical reactions, and it is also a measure of the potential for reactions, if chemical equilibrium between the water and surrounding rock has not been established. The pH of a water sample is a measure of the activity of hydrogen ions and is expressed in logarithmic units, with pH representing the negative base-10 log of the hydrogen ion activity in moles per liter. The pH of pure water at 25°C is 7.00, or neutral. Values less than 7.00 are acidic and values greater than 7.00 are basic, or alkaline. Dissolved gases such as CO2, SO2, and NO2 increase the number of hydrogen ions and cause waters to be acid. Carbonates, bicarbonates, hydroxides, phosphates, silicates, and borates decrease the number of hydrogen ions and cause waters to be basic. The hydrogen ion (H+) is generally the cause of acidity, and bicarbonate (HCO3–) is generally the source of alkalinity. Water with pH less than 6.5 is likely to be corrosive and have high iron and phosphate levels. Water with pH greater than 8.5 may result in turbidity from the precipitation of carbonate minerals, or high pH values may be the result of well drilling fluids remaining in the well after construction. Rainwater has a pH of about 5.5. As rainfall infiltrates into the ground, it reacts with carbon dioxide in the soil, resulting in low pH values typically measured in surficial aquifer wells. As water moves downward and interacts with aquifer minerals, acidity is consumed and alkalinity is produced, resulting in higher pH levels. For example, carbonate minerals such as calcite in the Floridan aquifer are highly reactive, raising pH levels.
Potassium
The principal sources of the potassium ion (K+) are the mixing of fresh groundwater with seawater in coastal transition zones and with connate water, and from the weathering of clays and feldspars. Weathering of potassium feldspars and clays is not considered a dominant process in Florida due to the scarcity of these minerals in aquifer sediments and slow weathering reaction rates. Potassium is rarely present in concentrations over a few milligrams per liter because potassium-rich sediments are scarce in the aquifer system and because potassium is immobilized as a nutrient by plants and sorbed onto clays.
Specific conductance
Specific conductance is a measure of the ability of water to conduct electric currents and is dependent on the concentrations and types of ions in the water and on temperature. Specific conductance has a strong positive correlation with TDS and with chloride concentrations. It is expressed in micromhos per centimeter at 25°C. There is a good statistical correlation between specific conductance and the total dissolved solids concentration and it can be used to approximate the dissolved-solids concentration of water.
Sodium
The primary sources of sodium (Na+) in Florida’s aquifer systems are the mixing of fresh groundwater with seawater along the coast and in coastal transition zones, upconing of seawater from deeper zones, the weathering of sodium-rich minerals such as clays or feldspars, and marine aerosols. Sodium-rich minerals are found in siliciclastic horizons of the surficial and intermediate aquifer system and minor amounts occur in the Floridan aquifer. The drinking water standard for sodium is 160 mg/L.
Sulfate
Sources of sulfate (SO42–) in groundwater include the dissolution of gypsum and anhydrite, the weathering of pyrite and iron sulfides, residual formation water, the lateral intrusion of seawater, precipitation that contains sulfur oxides, and marine aerosols. Sulfate is usually present in mine waters and some industrial waters. Sulfate in water containing calcium forms a hard scale in steam boilers; in large concentrations, sulfate in combination with other ions gives a bitter taste to water. The occurrence of sulfate depends upon the reduction/oxidation potential of the water. In reducing conditions, sulfate reduction produces hydrogen sulfide, which is the cause of the rotten egg odor in some wells. In oxidizing conditions, sulfides may be oxidized to sulfates. The drinking water standard for sulfate is 250 mg/L.
Temperature
The temperature of groundwater is controlled by climatic conditions, cultural activities, heat flow from the earth’s interior, and chemical reactions in the aquifer system. In shallow aquifers, water temperature is usually controlled by climatic conditions, as opposed to other possible causes. Water that has recently entered the aquifer system normally reflects atmospheric temperature at the time of recharge. In deeper aquifer systems, temperature can be affected by recharge from shallow environments, earth heat flow, and chemical reactions. Temperature measurements are recorded in the field.
Total dissolved solids
Dissolved solids in groundwater are the result of mineral dissolution reactions. Total dissolved solids (TDS) is a general measure of the total mass of ions dissolved in water and is usually determined from the weight of the dry residue remaining after evaporation of the volatile portion of an aliquot of the sample. Total dissolved solids values are widely used in evaluating and comparing water quality. Water that has recently entered an aquifer in recharge areas has had less time to dissolve minerals than has water that has traveled for some greater distance through the aquifer. TDS concentrations are higher for water from an aquifer comprised of reactive materials, such as limestone, than for an aquifer composed of quartz sand materials, which are relatively inert. TDS has a guidance criteria in groundwater of 500 mg/L. Water with a high TDS concentration may have an unpleasant taste, may contribute to the development of kidney stones, and may result in scale or precipitates in hot water heaters.
Total organic carbon
All natural waters contain organic material. The amounts present are small compared to the inorganic concentrations, but even small amounts can have significant effects on chemical properties. Organic carbon concentrations in groundwater are normally smaller than in surface waters.
Isotopes
An analysis of stable and radioactive isotopes is also provided for selected springs. Stable isotopes are used to identify sources of water and to learn more about hydrologic processes such as recharge, evaporation, mixing, and water-rock interactions. Radioactive isotopes are generally used for age-dating water because these isotopes decay over a period of time at a known rate.
Isotopes are atoms of the same chemical element that differ in mass because of a difference in the number of neutrons in the nucleus. There are two types of isotopes: stable and radioactive. Stable isotopes are used in hydrologic studies to identify sources of water and to learn more about hydrologic processes such as recharge, evaporation, mixing, and water-rock interactions. They are also useful in age-dating. Stable isotopes monitored for age-dating are helium-3, helium-4, and neon. Radioactive isotopes are generally used for age-dating water because these isotopes decay over a period of timeat a known rate. The radioactive isotopes monitored in age-dating are tritium and carbon-14.
Helium-3, Helium-4 and Neon
Concentrations of helium-3 and helium-4 can be used to determine an age for a water sample. Helium-3 is a radiogenic isotope of helium. It is produced by the radioactive decay of tritium. Both helium-3 and helium-4 are stable isotopes of helium. Helium-3 was measured to calculate the tritium/helium-3 age of the sample. Helium-4 was measured to correct the helium-3 concentration for contamination of helium-3 due to atmospheric sources — that is, helium-3 concentrations arising from equilibration with air during recharge and entrainment of air bubbles. Neon is a noble gas. Neon was measured to correct helium-4 for helium produced through the uranium and thorium decay series. The helium-3 in the water is assumed to be of atmospheric and tritogenic origin. This condition usually prevails in shallow aquifers containing predominantly young waters occurring in sediments and rocks of relatively low uranium and thorium content. Additional helium sources may be present in aquifers where the rocks are enriched in uranium or thorium, or in groundwater samples in which young water has mixed with relatively old water containing radiogenic helium. In these cases, the measured neon content can be used to calculate the additional helium.
Tritium
Tritium is a radioactive isotope of hydrogen that is produced naturally in small amounts by the interaction of cosmic rays with the earth’s atmosphere. Cosmogenic tritium enters groundwater by way of rainfall at a concentration of approximately 3 to 5 tritium units (TU; a tritium unit equals 3.2 picocuries/liter) (Kaufman and Libby 1954; Robertson and Cherry 1989). With the onset of atmospheric nuclear testing in 1953, the tritium concentration in rainfall began to increase. At Ocala, Florida, the tritium concentration in rainfall increased to as high as 700 TU in 1963. In 1988, the tritium concentration in rain at Ocala was not measurably different from the estimated pre-1953 concentration. Because of the difference in tritium concentration in rainwater before and after 1953, tritium has been used as a hydrologic tracer to date recent groundwater. The half-life of tritium is 12.43 years, which means that the tritium concentration decreases by one-half every 12.43 years. Tritium decays to Helium-3.
Tritium/Helium-3
The age determined by measuring the tritium and helium-3 concentrations in the sample is referred to as the piston flow age. It would be the age the water sample would have if the water traveled as a slug or “piston” through the aquifer.
Carbon-14
Carbon-14 is a radioactive isotope of carbon formed by the reaction between cosmic rays and nitrogen in the atmosphere. Carbon-14 combines with oxygen to form carbon dioxide, which is taken up by plants or adsorbed by rain and is found in surface water bodies. When plants, animals, and groundwater are no longer exposed to atmospheric carbon dioxide, the carbon-14 content begins to decline through radioactive decay. The radiocarbon content of groundwater decreases at a rate equal to the half-life of carbon-14, which is 5,730 years. Therefore, water that has been underground for an extended period of time will have a lower concentration of carbon-14 than water that has only recently entered the ground. The measured carbon-14 content of groundwater is expressed as a percentage of the modern carbon-14 content of groundwater, or percent modern carbon (pmc). Because the content of carbon-14 in the atmosphere increased after 1953, the base year for modern carbon-14 is 1950. The carbon-14 age is based on the Fontes and Garnier (1979) model for a system open to carbon dioxide. It represents the age for a leaky aquifer or an aquifer near a recharge area.
Carbon-13
Carbon-13 is a stable isotope of carbon. It normally is expressed with a delta notation which means it is measured with respect to a standard. The standard for carbon-13 is the PeeDee belemnite (PDB) or Vienna PDB carbonate standard. Carbon-13 is generally used to correct the measured concentration of carbon-14 for reactions along the flow path. Dissolution of limestone dilutes the concentration of carbon-14 in the system. Delta carbon-13 is used to correct for this dilution. Delta carbon-13 is generally near -12 in recharge areas and increases (becomes more positive) in discharge areas.
Laboratory water quality variables and field measurements
Variable | EPA Storet |
---|---|
Discharge, cfs | 61 |
Alkalinity, total, mg/L as CaCO3 | 410 |
Calcium, dissolved, mg/L as Ca | 915 |
Calcium, total, mg/L as Ca | 916 |
Chloride, total, mg/L as Cl * | 940 |
Fluoride, dissolved, mg/L as F | 950 |
Fluoride, total, mg/L as F | 951 |
Magnesium, dissolved, mg/L as Mg | 925 |
Magnesium, total, mg/L as Mg | 927 |
Nitrate + nitrite, total, mg/L as N * | 630 |
Nitrate + nitrite, dissolved, mg/L as N | 631 |
Orthophosphate, total, mg/L as P * | 70507 |
pH, field | 400 |
Phosphorus, total, mg/L as P | 665 |
Potassium, dissolved, mg/L as K | 935 |
Potassium, total, mg/L as K | 937 |
Sodium, dissolved, mg/L as Na | 930 |
Sodium, total, mg/L as Na | 929 |
Specific conductance, field, µmhos/cm at 25 °C | 94 |
Specific conductance, lab, µmhos/cm at 25 °C | 95 |
Sulfate, total, mg/L as SO4 * | 945 |
Total dissolved solids, mg/L * | 70300 |
Total organic carbon, mg/L as C | 680 |
Water temperature, °C | 10 |
Units: µmhos/cm = micromhos per centimeter
mg/L = milligrams per liter
cfs = cubic feet per second
* Data for these variables are graphed and analyzed for trends if spring sampled at least 8 times
Brooks, H.K. 1961. The submarine spring off Crescent Beach, Florida. Quart. J. Fla. Acad. of Sci. 24(2):122—34.
Davis, J.B. (in review). Aerial thermography survey and seismic reflection profiles from the Silver River, Marion County, Florida. St. Johns River Water Management District, Palatka, Fla.
[DEP] Florida Department of Environmental Protection. 2000. Florida’s springs — strategies for protection and restoration. Tallahassee, Fla.
Ferguson, G.E., C.W. Lingham, S.K. Love, and R.O. Vernon. 1947. Springs of Florida. Bulletin 31. Tallahassee: Florida Bureau of Geology.
Fontes, J.C., and J.M. Garnier. 1979. Determination of the initial 14C activity of the total dissolved carbon: A review of the existing models and a new approach. Water Resources Research 15(2):399—413.
Gilbert, R.O. 1987. Statistical methods for environmental pollution monitoring. New York: Van Nostrand Reinhold.
Helsel, D.R., and R.M. Hirsh. 1992. Statistical methods in water resources.Elsevier, N.Y.: Elsevier Science B.V.
Kaufman, S., and W.F. Libby. 1954. The natural distribution of tritium. Phys. Review93:1337—44.
Kindinger, J.L., J.B. Davis, and J.G. Flocks. 2000. Subsurface characterization of selected water bodies in the St. Johns River Water Management District, northeast Florida. U.S. Geological Survey Open-File Report 00-180.
Odom, H.T. 1957. Trophic structure and productivity of Silver Springs, Florida. Ecological Monographs, Vol 27, No 1.
Osburn, W., D. Toth, and D. Boniol. 2002. Springs of the St. Johns River Water Management District. Technical Report 2002-5. Palatka, Fla.: St. Johns River Water Management District.
Phelps, G.G. 2004. Chemistry of ground water in the Silver Basin, Florida, with an emphasis on nitrate. U.S. Geological Survey Scientific Investigations Report 2004-5144.
Puri, H.S., and R.O. Vernon. 1959. Summary of the geology of Florida and a guide to the classic exposures. Special Publication 5. Tallahassee: Florida Geological Survey.
Robertson, W.D., and J.A. Cherry. 1989. Tritium as an indicator of recharge and dispersion in a groundwater system in central Ontario. Water Resources Research 25(6)1097—1109.
Rosenau, J.C., G.L. Faulkner, C.W. Hendry Jr., and R.W. Hull. 1977. Springs of Florida (revised). Bulletin 31. Tallahassee: Florida Bureau of Geology.
Scott, T.M., G.H. Means, R.P. Meagan, R.C. Means, S.B. Upchurch, R.E. Copeland, J. Jones, T. Roberts, A. Willet. 2004. Springs of Florida (revised). Bulletin 66. Tallahassee: Florida Geological Survey.
Spechler, R. M. 1996. Detection and quality of previously undetermined Floridan aquifer system discharge to the St. Johns River, Jacksonville to Green Cove Springs, northeastern Florida. U.S. Geological Survey Water Resources Investigations Report 95-4257.
Swarzemski, P.W., C.D. Reich, R.M. Spechler, J.L. Kindinger, and W.S. Moore. 2001. Using multiple geochemical tracers to characterize the hydrogeology of the submarine spring off Crescent Beach, Florida. Chemical Geology 179:187—202.
Toth, D.J. 1999. Water quality and isotope concentrations from selected springs in the St. Johns River Water Management District. Technical Publication SJ99-2. Palatka, Fla.: St. Johns River Water Management District.
Toth, D.J., and C. Fortich. 2002. Nitrate concentrations in the Wekiva groundwater basin with emphasis on Wekiwa Springs. Technical Publication SJ2002-2. Palatka, Fla.: St. Johns River Water Management District.